Advances in external beam stereotactic body radiotherapy: principle concerns in implementing a liver radiation program
Introduction
Liver cancer is a growing worldwide concern and is the second most common cause of death from cancer worldwide (1). Data that is under continual development through a collaboration of the GLOBOCAN Project, Cancer Today, International Atomic Energy Agency and the World Health Organization continue to demonstrate the disproportionate increase and poor prognosis, and impact on less developed countries, relative to other cancers. Eighty-three percent of cases occur in less developed regions with 50% in China alone. Prognosis remains poor with an overall ratio of mortality to incidence of 0.95. Therefore, geographical death rates are correlated with incidence with most deaths worldwide occurring in China followed by sub-Saharan Africa, then Japan and South-East Asia (2). In Canada, rates rose in men between 40–84 years of age from an incidence of 5.4 per 100,000 to 15.4 per 100,000 between the periods of 1976–1980 and 2006–2010 (3). Secondary hepatic malignancies account for a majority of liver lesions especially from colorectal carcinoma. Liver involvement remains a poor prognostic factor with 50% of patients diagnosed with metastases at diagnosis or at recurrence (4).
Given this burden and prognosis, investigation into improving detection and treatment is considered a priority research and management area. Important advances have been made such as promotion of guidelines to increase early detection in high risk populations such as screening at 6 month intervals by the American Association for the Study of Liver Diseases (5). In a landmark cluster randomization study in China, a 37% relative reduction in liver related mortality was achieved with ultrasound and alpha fetoprotein assessment alone (6). In addition, improved surgical techniques such as associating liver partition and portal vein ligation for staged hepatectomy (ALPPS) that are attempting to improve the number of patients eligible for surgical resection (7). Systemic treatment now has high level evidence for first line use of sorafenib [based on the Hong Kong (8) and SHARK (9) investigators] and second line with regorafenib and ramucirumab (10). However, these studies remain pertinent to a select group of patients with many frequent exclusionary criteria plus they have relatively small impacts on overall survival. For this reason, centres are seeking new treatments and establishing specialized multidisciplinary teams. A combination of established treatments, investigation of new treatments and/or sequencing of treatments amongst several disciplines remains an active area of investigation in the hopes of improving outcomes. With the continued increase in availability and clinical experience of stereotactic body radiotherapy (SBRT), we now have non-randomized evidence of benefit and minimal toxicity in both primary and secondary liver lesions (11,12). Though there remains a large variability in technique, patient selection, and reported outcomes, there is a convergence to a standard radiotherapy process (13). Centres hoping to initiate programs or with established programs face a lack of level I evidence. Implementation requires a significant change in management process and philosophy. For example, radiation technologists must learn new image guidance skills and physicians need to use new tools to guide the appropriate selection of eligible patients and dose to avoid life threatening toxicities. Centres starting programs or aiming to improve or standardize techniques seek data on safety, appropriate dose selection, protocols to allow for efficient implementation, methods to deal with common problems and practical implementation processes.
Toxicity
In 1924, the first reported trial of radiation of the human liver lead to a belief that liver cells were resistant to radiation (14). Early trials such as this also established clinical and pathologic evidence that radiation of the liver caused hepatitis sometimes leading to death (15). Therefore, radiation has historically been contraindicated due to the risk of serious harm and the belief that there is minimal benefit due to inability to reach tumorocidal doses. When first starting liver radiation programs, there may be resistance to implementation and reluctance to refer from other disciplines. Therefore, a detailed understanding of current technology and experience is needed to implement a program.
Radiation induced liver disease (RILD) is a constellation of signs formalized as any one of the following within 3 months of radiation: (I) transaminase or alkaline phosphatase elevation greater than 2.5–5 times normal; (II) bilirubin elevation of greater than 1.5–3 times normal or pretreatment level; and (III) non-malignant ascites in the absence of disease progression (16). Central venous congestion and collagen deposition causing small vein obstruction without inflammation is seen pathologically. This distinguishes this from non-radiation induced hepatitis which is often also present. Palliative treatments include diuretics, paracentesis, and vitamin K. Fortunately, there has been significant research to address these concerns. The North American research can be represented by the publication history of two groups (University of Toronto and Indiana University). The Indiana University has provided safety data based on a step-wise dose escalation program (17). Indiana University escalated the dose from 36 Gy in 3 fractions in 2 Gy/fraction steps. For the Child-Pugh (CP)-A cohort, they were able to escalate to 48 Gy in 3 fractions without any dose limiting toxicity (DLT), defined as greater than grade 3 Common Terminology Criteria for Adverse Events v3.0. However, for the CP-B cohort, 2 of the 17 patients developed DLT. Therefore, Indiana University subsequently instituted a slightly more protracted regimen for CP-B patients of 40 Gy in 5 fractions and they have experienced no further cases of RILD in over 60 cases with sufficient median follow up of 27 months. (18) Therefore, if one applies the Indiana University recommendations of differential dosing based on CP score (CP < B8), and constrain 700 cc of normal liver to receive less than 15 Gy, the chance of RILD is extremely unlikely. The University of Toronto group provided data using a radiobiologically-guided partial volume dose escalation program (19). In their first report, 41 patients received doses from 24–54 Gy in 6 fractions daily. Patients had to have more than 700 cc of normal liver. Toxicities reported was one case of grade 3 nausea and vomiting, 8 cases of transaminitis (6 of whom had similar elevations prior to radiation) and 5 cases had a decline of their CP scores. Multiple studies have since been published that have demonstrated the safety of partial liver irradiation using this type of careful dose selection by CP score and specific constraints sparing normal liver (20).
Therefore, RILD may not represent the limiting factor for implementation of radiotherapy of the liver. Several other non-RILD toxicities exist, including gastroduodenal damage, chest wall and rib injury, coagulopathies, esophageal ulceration, renal failure, reactivation of viral hepatitis, cardiac injury, pneumonitis and skin necrosis. Of these, a review of the literature suggests that gastroduodenal toxicity may be the limiting factor and source of the most concerning toxicities. Indeed, in the trial from the University of Toronto described above, one patient developed duodenal bleeding which was fatal (19). A further three patients experienced bowel obstruction or transient biliary obstruction in the 49 patient sample. This is not unique to hepatic irradiation. In one of the largest series of abdominal SBRT, Bae found that 15% of patients developed greater than or equal to grade 2 gastroduodenal toxicity (21). Of 202 patients, he found 40 patients with gastroduodenal segment proximity that received a dose greater than 11 Gy. The median time to toxicity was 6 months and a clinical history of ulcers or cholangiocarcinoma was a strong predictor of toxicity. This study provides predictive parameters including data that indicates relative safety (defined as 5% risk of toxicity) if Dmax is kept below 35 Gy. Note that there is a steep increase beyond this dose; Bae found a 10% risk when the maximum dose rises to 38 Gy.
The current literature on safety is based on case series level evidence with studies containing heterogeneous populations and treatments. With that caveat, the data does provide good support that radiation of liver lesions can be safe and well tolerated. With methods to limit the risk of RILD, gastroduodenal toxicity may be the greatest concern to radiation oncologists. The literature provides specific dosimetric constraints to ensure the safe implementation of liver radiation summarized in Table 1.
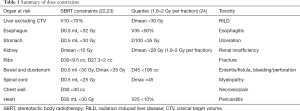
Full table
Dose selection and outcome
Two independent and comprehensive surveys found a large variation in dose selection (13,25). In the 2012 study, the three most common regimens for radical liver treatment were 45 Gy in 3 fractions, 45 Gy in 15 fractions, and 40–50 Gy in 5 fractions. Since then there have multiple case series providing information on dose escalation and selection. These trials suggest that there is a difference in dose required to control primary versus secondary malignancies (25). The TD50 (tumour dose required to achieve a 50% control rate) was 53 Gy EQD2 (2 Gy equivalent dose) for primary hepatic malignancies; a higher TD50 of 70 Gy EQD2 was required for metastatic disease. Furthermore, the trial by Lausch et al. demonstrated that local control probability was dose dependent in a sigmoidal pattern for both groups. This was consistent with preclinical data using mathematical modeling of tumour response to radiation (26). To achieve a TD90, primary hepatic carcinoma was shown to be more sensitive than secondary malignancies and a dose of 84 Gy EQD2 could achieve a 90% 6-month local control rate; secondary hepatic lesions would require 95 Gy EQD2 to achieve the same local control.
In an evidence-based review supported by ASTRO, CARO, ESTRO and TROG/RANZCR, Høyer et al. suggested that a dose of 48 Gy in 3 fractions would be a suitable standard dose for liver metastases (11). However, Rule et al. (27) have conducted a formal dose escalation cohort program. In this program, the 2-year actuarial local control rose from 56% to 100% when the dose was escalated from 30 Gy in 3 fractions to 60 Gy in 5 fractions. Given the safety and remarkable control rate they achieved, 60 Gy in 5 fractions could be considered as a new standard, normal tissue tolerance permitting. However, many factors play a role in dose selection and reported outcomes. A review of dose escalation studies (Table 2 representative dose escalation studies, equivalent doses and outcomes) suggests that those patients that are heavily pre-treated with chemotherapy have more resistant disease and may require higher doses as is suggested in the two reports from the London Regional Cancer Program (25,33); studies such as the one by Rule et al. (27) included patients with smaller lesions and healthier patients which may explain a higher control rate and ability to achieve high doses. Many patients with larger lesions or within close proximity to dose limiting normal structures, may not be able to tolerate 60 Gy. Aside from this variability, all trials seemed to achieve high rates of control with minimal toxicity (11). Therefore, a practical approach would be to attempt to dose escalate with the goal of achieving a 60 Gy in 5 fractions equivalent dose and thereby achieve the highest chances of local control demonstrated in the literature. Patients that are unable to achieve the constraints of this dose regimen can attain a similar response at an equivalent of 48 Gy in 3 fractions regimen. Conversely, one must be aware that there may be a threshold below which local control decreases steeply. In 2009, McCammon et al. (34) demonstrated a 3-year local control of 59–89%, but this dropped to 8% if doses were below 36 Gy. This group, recommended attempting to achieve a dose greater than 65.3 Gy EUD. Another method to select dose is a radiobiologically guided dose selection algorithm. This method is used to individually select the maximum dose possible for each patient with specific toxicity risk levels (19,31). This is the technique used at our centre and is described below. For hepatocellular carcinoma dose selection, a similar consensus within the literature has been reached. In a dose escalation landmark trial published by Cárdenes et al. (17), dose was escalated to 48 Gy in 3 fractions at a maximum of two treatments per week. Patients with CP-B7 or greater receive a reduced dose of 40 Gy in 5 fractions. This lowered dose is not only safer, but there is data to suggest that patients with underlying liver insufficiency do not benefit from dose escalation (33) With these trials guiding dose selection, a review of the data indicates that we can achieve a 2-year local control rate of 60–90% for liver metastases (11); and a 2-year local control rate of 80–90% and 1-year overall survival of 48–93% for primary liver lesions (12,20).
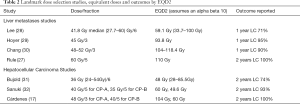
Full table
Components of liver SBRT
Technological advances in imaging, treatment planning, image-guidance, respiratory motion management, and beam delivery, have allowed us to consider treating liver tumours with higher doses of radiation. However, compared to lung SBRT, liver SBRT is still evolving. Current issues include, but are not limited to: (I) difficulty in tumor localization due to low CT contrast between the tumor and surrounding normal liver; (II) lack of consensus on prescription dose; (III) lack of consensus on beam delivery technique [conventional fixed-beam 3D conformal radiotherapy vs. intensity-modulated radiation therapy (IMRT) vs. arc therapy]; and (IV) lack of consensus on the use of respiratory motion management techniques and the significant increase in respiratory motion of the tumor compared to other extra-cranial sites. To effectively treat liver tumors with ablative radiation doses, increased attention on the accuracy of the entire treatment planning and delivery process must be considered. Therefore, a practical approach that draws on available data and grass roots experience may assist in implementing or standardizing a safe and efficient liver SBRT program.
Respiratory motion management
One of the largest sources of uncertainty in radiotherapy of cancers in the thorax and abdomen, is the impact of respiratory motion during both imaging and radiation delivery. Lung tumours, for example, have been shown to move up to 5 cm with free breathing (35). The magnitude of motion, at the same time, is variable and unpredictable (36,37). Liver tumours have been shown to move significantly more than lung tumours due to the influence of the diaphragm (38-42). Assessing the motion of a tumour and nearby critical structures during respiration is essential for accurate imaging, treatment planning, patient setup, and dose delivery. AAPM Task Group 76 provides a detailed description of respiratory motion management techniques in situations where tumor motion exceeds 5 mm (43). These techniques are classified into: (I) motion encompassing methods; (II) voluntary/involuntary breath-hold methods; (III) tumor immobilization methods; (IV) respiratory gating methods; and (V) tumor tracking methods. The treatment planning process for liver radiotherapy can vary depending on the choice of respiratory motion management technique as described below.
Simulation imaging
As with SBRT of other extra-cranial sites, precise delineation of the target and surrounding patient anatomy is crucial. Since modern radiotherapy treatment planning still relies on 3D-CT for inferring electron density required for radiation dose calculation, CT remains as the primary imaging modality for liver SBRT. However, due to low CT contrast between the tumor and surrounding normal liver, delineation of the target remains a primary uncertainty in the treatment planning process (44). Multi-modality imaging such as dynamic contrast-enhanced CT, magnetic resonance imaging (MRI), and positron emission tomography (PET) have been shown to effectively localize tumors in the liver, if the imaging is performed with the patient in the treatment position (45-47). Unfortunately, many centers either do not have timely access to multi-modality imaging or do not get imaging in the planning position. Uncertainties due to deformation and respiratory motion have also deterred centers from including multi-modality imaging.
The ability of a fast, multi-slice helical CT scanner to accurately predict the expected target position during beam delivery suffers under normal respiration as it merely takes a snapshot of the tumor, and may not represent its mean position. The consequence is placing large arbitrary margins to define the planning target volume (PTV) which may expose more normal tissue to high radiation dose. The most common techniques used to better characterize the expected tumor motion during treatment delivery include slow CT, breath-hold CT, prospective gated techniques, and four-dimensional CT (4D-CT).
4D-CT imaging has become a staple for providing 3D dynamic anatomical information used in modern radiotherapy of mobile tumours that are influenced by respiratory motion (48-52). Such information can be used to define an internal target volume (ITV) that accounts for all internal motion, including the respiratory motion encompassment of the tumour. A subset of the 4D-CT dataset can also be used for respiratory-gated radiation therapy where the radiation is turned on only during a predefined portion of the respiratory cycle, minimizing the tumour motion during delivery (53). A 4D-CT dataset is composed of a time series of 3D images at multiple phases of the patient’s breathing cycle. This is accomplished by acquiring multiple projections of the same anatomical space under free breathing conditions and retrospectively binning either the projection data, acquired with a low-pitch helical CT scan, or sequentially acquired 2D axial images, with the couch fixed (cine mode) according to a breathing trace that was acquired simultaneously. Conventional multi-slice CT scanners have an axial field-of-view (FOV) up to 4 cm necessitating scanning over multiple respiratory cycles to cover the intended scan range, even though each axial slice location is imaged for only one respiratory cycle. As a result, the quality of the 4D-CT dataset relies heavily on the patient’s ability to breathe reproducibly, the respiratory surrogate, and the CT sorting algorithm that may combine volumes from temporally misaligned phases into a single volume. Many methods have been proposed to improve 4D-CT sorting methods for irregular breathing patients, including phase tagging adjustment (48,50,51), amplitude-based sorting methods (54-57), retrospective waveform manipulation (58-60), and non-linear image registration (61-64). Despite these improvements, motion artifacts can also be induced due to poor correlation between the motion of the internal anatomy and that of the respiratory surrogate (65,66). Accuracy of target volume and normal tissue segmentation, dose calculation, and treatment setup registration accuracy can and have been known to be affected by respiratory motion artifacts (67-71). If we do not account for these uncertainties, we increase the risk of geographic miss of the target and unintended increase of radiation dose to normal tissues; both of which could impact overall survival.
Despite the increased world-wide use of 4D-CT imaging for radiotherapy of mobile tumors, the ability to localize liver tumours still suffers due to low CT contrast between the tumour and surrounding normal liver. Contrast enhanced 4D-CT has been proposed to increase the delineation accuracy of liver lesions affected by respiratory motion. However, one must be aware of the dual-blood supply from the hepatic portal vein and the hepatic arteries. The hepatic portal vein supplies 75% of the blood to the liver, while the hepatic arteries supply the remaining 25%. Most primary hypervascular hepatocellular (HCC) enhance during the arterial phase of the contrast scan while metastatic hypovascular colorectal metastases are best visualized during the portal-venous phase. The washout/equilibrium phase is used to visualize fibrotic lesions such as HCCs and cholangiocarcinomas (44,72-75) . Since 4D-CT scans require long acquisition times, it is impossible to detect all phases of contrast enhancement at the same axial location.
Respiratory compensated tri-phasic contrast enhanced CT imaging is a method that can increase the delineation accuracy of the target. Two end exhale breath-hold fast multi-slice CT scans, acquired at 30 and 70 seconds post contrast injection represent the arterial and portal venous phases, respectively. At 150 seconds post injection, a 4D-CT scan is acquired during the washout phase. The end exhale breath-hold scans are then registered to the end exhale phase(s) of the 4D-CT dataset for tumour delineation. Figure 1 shows the respiratory trace generated from the Varian Real-Time Position Management (RPM) device (Varian Medical Systems, Palo Alto, USA) during a tri-phasic imaging session of one liver patient. Figure 2 shows the corresponding images reconstructed at each imaging phase.
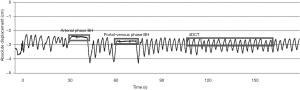
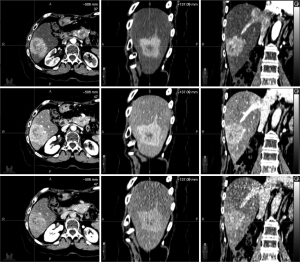
At minimum, simulation should involve the acquisition of a 4D-CT via a system composed of a fast, multi-slice CT scanner, a method to simultaneously generate a respiratory trace, and a method to retrospectively sort CT data into multiple respiratory bins (or phases). Additional imaging methods to improve localization in low CT contrast areas should be considered, including contrast-enhanced CT or MRI. The report of the AAPM Task Group 76 describes multiple methods, including advantages and disadvantages of each technique, to image moving targets (43).
Target volume delineation
The goal of radiation therapy is to deliver a high radiation dose to a target containing the gross-tumour, while keeping the volume of normal tissue receiving high doses outside the target as low as possible to minimize the risk of toxicity. For SBRT of liver tumours, it is imperative to have the high dose of radiation fall-off sharply outside the target volume to minimize the damage to normal liver. The International Commission on Radiation Units and Measurements (ICRU) Reports 50 (76) and 62 (77) definitions of the gross tumour volume (GTV), clinical target volume (CTV), and PTV can be utilized, however, the ITV depends on the respiratory motion management technique that will be applied during beam delivery.
ITV for motion encompassing methods
In this classification, the ITV can be extracted from the 4D-CT dataset. This can be accomplished by: (I) delineating the GTV directly on the average of the 4D-CT dataset. Often this dataset lacks the spatial resolution required to accurately delineate the GTV; (II) delineation of the GTV on each phase of the dataset, followed by adding a CTV margin to each GTV, and creating an envelope of each CTV; and (III) delineating the GTV at end inhalation and end exhalation, followed by adding a CTV margin to both GTVs, and creating an envelope of both CTVs. It is imperative that the path of the target during inhalation is similar to the path during exhalation. Any difference (often referred to as hysteresis) should be accounted for in the ITV. Motion encompassing methods are often discouraged in liver SBRT as they significantly increase the dose to normal liver. As a result, motion encompassing methods can limit potential dose escalation to the target. Such an approach should be used only if the motion is not significant or if the patient cannot comply with other motion management techniques.
ITV for breath-hold and tumour immobilization techniques
In these classifications, CT simulation must be performed under the same conditions. For example, breath-hold techniques that are applied during beam delivery should be simulated during CT scanning. This may include additional breath-hold CT scans. Then the classical definitions of GTV, CTV, and PTV defined in ICRU 62 should be followed. However, the uncertainties involved in reproducing the breath-hold should be accounted for in the ITV. For example, the depth of end-exhale breath-hold may drift with time and repeated breath-holds. Similar approaches to ITV construction can be applied to tumor immobilization methods, such as abdominal compression.
ITV for respiratory gating techniques
Respiratory gating methods are beneficial to those who cannot comply with breath-hold or tumour immobilization techniques, and whose tumor motion is deemed too high to avoid over treating normal tissues. In this case, either a single phase from the 4D-CT (for example, end exhalation) dataset can be used for delineation of the GTV. Again, the classic definitions of the CTV and PTV can be employed. However, residual motion that occurs during the gating window (the portion of the respiratory cycle where the beam is turned on) must be added to the CTV to define the ITV. Alternatively, phases of the 4D-CT dataset that fall within the intended gating window can be averaged together to form a single 3D-CT dataset. The advantage to using this dataset for GTV delineation include direct incorporation the residual motion of the tumour on a dataset that has decreased image noise compared to using a single phase of the 4D-CT dataset. Figure 3 compares the subset average CT scan to the end exhale CT scan. The greater information available from this subset average CT provides better image quality needed for image guidance.
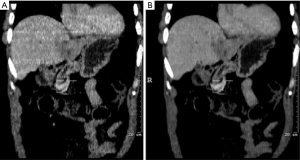
ITV for tumor tracking techniques
Tumour tracking methods are also advantageous to patients who cannot comply with breath-hold or tumour immobilization techniques. Also, unlike respiratory gating, the beam is on during the entire treatment delivery. The ITV in this case does not include an expansion to account for the motion extent, but should still account for rotations and deformations of the target during respiration.
As per the recommendations of the AAPM Task Group 76, any respiratory motion management technique should be used when tumor motion is deemed significant (>5 mm). Careful attention must be taken when delineating the GTV, CTV, ITV, and PTV as accurate definition depends on the technique used.
Dose prescription
Despite the technical advances in imaging, radiation treatment planning, image-guidance, and beam delivery, there still remains a lack of consensus on the dose prescription for treating both HCCs and liver metastases. SBRT has shown to be effective in obtaining a treatment response in most patients and a dose as high as 60 Gy in 5 fractions has been proposed (34). However, patients were highly preselected and eligibility depended on the size of the target and its vicinity to sensitive organs at risk (OAR). For this reason, radiobiologically-based dose prescriptions based on normal tissue complication probability (NTCP) have been proposed (78).
NTCP based dose prescription
NTCP models have been used to determine the risk of RILD (79-81).These models can be used to individualize the dose prescription to levels as high as possible while maintaining the NTCP below a desired threshold. The Lyman-Kutcher-Burman NTCP model has been used to predict the risk of causing liver complication. This model is based on a sigmoidal dose-response relationship. The NTCP model takes into account the magnitude of volume effect for the liver based on serial or parallel organ classification (n), the steepness of the dose-response curve for a specified partial volume (m) and the tolerance dose for the whole liver that causes 50% complication (TD50). For primary HCCs, we use (n=1.1, m=0.18, and TD50 =43.4 Gy) and for metastatic disease we use (n=0.97, m=0.12, and TD50 =39.8 Gy). These parameters were based in part on a prospective study of 203 patients treated at 1.5 Gy per fraction and used to validate the LKB models (80). Depending on the fractionation used, the dose-volume histogram is normalized to 1.5 Gy per fraction based on an α/β=2.5 for liver. Then the DVH is reduced to the effective irradiated liver volume, Veff. Once a treatment plan is optimized for an arbitrary prescription, the prescription dose is then escalated until either the NTCP of the normal liver exceeds 5% or physical dose constraints are exceeded.
Treatment planning
Recent advancements such as IMRT, volumetric modulated arc therapy (VMAT), and helical tomotherapy (HT) has allowed for better conformality of the PTV and with SBRT dose delivery techniques, have allowed for sharp dose fall-off outside the PTV. Hence, the ability for dose escalation within the target volume has restored the interest in radiation therapy as a radical modality for liver malignancies. The aforementioned techniques have facilitated dose escalation and offer other clinical gains such as higher minimum dose to the PTV and reduction in dose to OARs. These techniques have been implemented in numerous clinics for the treatment of liver cancer.
For example, the Varian TrueBeamTM linear accelerator (Varian Medical Systems, Palo Alto, CA, USA) allows for VMAT delivery, with and without respiratory gating, dose rates as high as 2,400 MU/min when using flattening filter free (FFF) beams, and during treatment kV imaging capabilities for beam delivery verification. Rather than assigning a standardized dose prescription, dose-escalation can be used to determine the highest dose achievable while maintaining tolerance doses of critical structures. Dose constraints are shown in Table 1 and are based on the Radiation Therapy Oncology Group (RTOG) 1112 (23) and a comprehensive review of SBRT for gastrointestinal organs (22). Various high precision techniques are available. Arc based radiotherapy is particularly useful from the point of view of radiobiology and time required for treatment. Figure 4 compares a VMAT plan versus a 9 beam IMRT plan. Both plans were optimized with the Pinnacle Treatment Planning System v9.6 (Philips Medical Systems, Fitchburg, USA). Even though arc-based delivery techniques increase the volume of low dose, the impact on the NTCP seems unaffected. The ability to decrease the intermediate dose compared to fixed beam IMRT allows for a decrease in the NTCP and hence, a higher prescription dose. Lastly, treatment planning without a pre-determined dose can be difficult. A graphical plot (Figure 5) of the normal liver NTCP versus prescription dose can help treatment planners choose an appropriate maximum escalated prescription dose. This maximum dose can be visually determined as the elbow of the curve before small increments in prescribed dose would result in exponential increases in toxicity risk. Here, it is evident that the patient can receive a dose higher than 14 Gy/fraction (or 84 Gy in 6 fractions) with this treatment technique while maintaining a liver NTCP less than 5%.
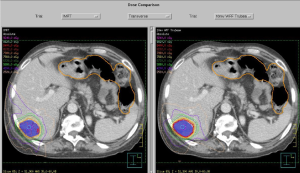
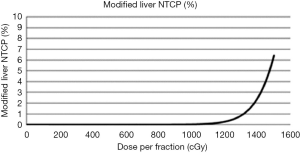
Image-guidance
In room fluoroscopy, 2D (megavoltage) MV or (kilovoltage) kV imaging, stereoscopic X-rays, and cone-beam CT (CBCT) are all methods that can be used localize targets. However, for liver cancer radiotherapy, poor tumour visualization and significant respiratory motion make it difficult using currently available methods. For breath-hold and tumour immobilization techniques, CBCT can be acquired under the same motion management position. Calcifications, surgical clips, implanted markers, or contrast (such as lipiodol) can be used to align the liver from the CBCT to the planning CT. For free breathing, non-gated treatments, free breathing CBCT scans can be advantageous due to the “smearing” of the liver. This volume can be aligned with the average 4D-CT dataset used for radiotherapy planning. Alternatively, if the end exhale phase of the 4D-CT dataset was used for radiotherapy dose calculation, then with proper window/leveling, the dome of the diaphragm that is visible on the free breathing CBCT will appear at its most superior location representing the end exhale phase. Some linear accelerators have 4D-CBCT capabilities which can allow for the full respiratory motion extent to be aligned with the average 4D-CT dataset. However, not all vendors offer this option clinically for on-line image-guidance.
For patients that receive respiratory gated radiotherapy, multiple on-line image guidance procedures can be used. For centers without 4D-CBCT capabilities, a free breathing CBCT can be acquired where the superior aspect of the “smeared” liver volume represents the end exhale position can be aligned to the end exhale phase of the 4D-CT or the subset average CT if also acquired at end exhale. Figure 6 demonstrates the application of image guidance where a subset average CT near end exhalation is matched to a free breathing CBCT. In contrast to ungated conditions, this setup verification method does not validate the gating window. For example, a free breathing CBCT dataset does not reflect nor can detect if there is a phase shift between the external marker motion and the liver/diaphragm motion. Orthogonal kV fluoroscopic image guidance or respiratory gated 2D kV images can be used in conjunction with CBCT imaging to verify the gating window, while ensure 3D patient setup verification. Figure 7 illustrates 2D gated kV matching at end exhalation with a gated digital reconstructed radiograph, also at end exhalation.
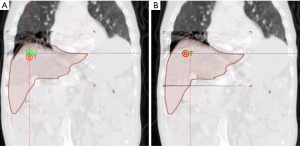
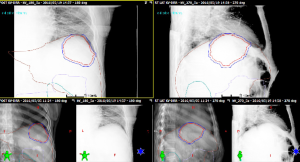
Discussion
A successful liver SBRT program is one that comprehensively considers patient selection, precise and accurate imaging, advanced treatment planning optimization, image-guidance, respiratory motion management, and precise radiation dose delivery. There are numerous methods for achieving quality radiotherapy for this patient population, and extreme caution must be taken at specific stages of the treatment planning/delivery process. The suggested approach to management starts first with appropriate patient selection. Multidisciplinary input is important particularly as there is growing evidence that combination and/or sequencing treatments results in better results. Dose selection, if not radiobiologically guided, is converging on 48 Gy in 3 fractions equivalent (or 40 Gy in 5 fractions for those with worse than CP-B7 liver function). Liver metastases are more resistant and there is good data to suggest escalation to 60 Gy in 5 fractions. Adherence to strict patient selection criteria and dosing based on available data from centres with reported serious toxicities is critical in an area without randomized evidence. Treatment constraints (Table 1), based on these case series, provide significant confidence regarding safety of liver radiation.
Conclusions
There is increasing acceptance and implementation of radiation for liver malignancy. There has been increased standardization of procedures and a better understanding of parameters that can assist in dose selection and prediction of outcome. Technical management details presented here may provide a path to more efficient implementation and standardization of radiotherapy for patients with few options.
Acknowledgements
The authors would like to thank Sarah Freiburger for proofreading the manuscript and for her helpful suggestions.
Footnote
Conflicts of Interest: MI Lock has received fees as a consultant or research funding from AstraZeneca Limited, Accuray Incorporated, 3M Canada, Varian Medical Systems and Abbvie Corporation. The other author has no conflicts of interest to declare.
References
- Ervik M, Lam F, Ferlay J et al. Cancer Today. Int Agency Res Cancer 2016. Available online: http://gco.iarc.fr/today
- Ferlay J, Soerjomataram I, Ervik M, et al. GLOBOCAN 2012 v1.1, Cancer Incidence and Mortality Worldwide: IARC CancerBase No. 11. Lyon, France: International Agency for Research on Cancer, 2014.
- Pocobelli G, Cook LS, Brant R, et al. Hepatocellular carcinoma incidence trends in Canada: Analysis by birth cohort and period of diagnosis. Liver Int 2008;28:1272-9. [Crossref] [PubMed]
- Bosch FX, Ribes J, Díaz M, et al. Primary liver cancer: Worldwide incidence and trends. Gastroenterology 2004;127:S5-16. [Crossref] [PubMed]
- Bruix J, Sherman M. American Association for the Study of Liver Diseases. Management of hepatocellular carcinoma: an update. Hepatology 2011;53:1020-2. [Crossref] [PubMed]
- Zhang BH, Yang BH, Tang ZY. Randomized controlled trial of screening for hepatocellular carcinoma. J Cancer Res Clin Oncol 2004;130:417-22. [Crossref] [PubMed]
- Schadde E, Ardiles V, Robles-Campos R, et al. Early survival and safety of ALPPS: first report of the International ALPPS Registry. Ann Surg 2014;260:829-36. [Crossref] [PubMed]
- Cheng AL, Kang YK, Chen Z, et al. Efficacy and safety of sorafenib in patients in the Asia-Pacific region with advanced hepatocellular carcinoma: a phase III randomised, double-blind, placebo-controlled trial. Lancet Oncol 2009;10:25-34. [Crossref] [PubMed]
- Llovet JM, Ricci S, Mazzaferro V, et al. Sorafenib in advanced hepatocellular carcinoma. N Engl J Med 2008;359:378-90. [Crossref] [PubMed]
- Zhu AX, Baron AD, Malfertheiner P, et al. Ramucirumab as Second-Line Treatment in Patients With Advanced Hepatocellular Carcinoma: Analysis of REACH Trial Results by Child-Pugh Score. JAMA Oncol 2016. [Epub ahead of print]. [PubMed]
- Høyer M, Swaminath A, Bydder S, et al. Radiotherapy for liver metastases: A review of evidence. Int J Radiat Oncol Biol Phys 2012;82:1047-57. [Crossref] [PubMed]
- Klein J, Dawson LA. Hepatocellular carcinoma radiation therapy: review of evidence and future opportunities. Int J Radiat Oncol Biol Phys 2013;87:22-32. [Crossref] [PubMed]
- Lock MI, Hoyer M, Bydder SA, et al. An international survey on liver metastases radiotherapy. Acta Oncol 2012;51:568-74. [Crossref] [PubMed]
- Case JT, Warthin AS. The Occurrence of Hepatic Lesions in Patients Treated by Intensive Deep Roentgen Irradiation. Amer J Roentgen 1924;12:27-46.
- Lawrence TS, Robertson JM, Anscher MS, et al. Hepatic toxicity resulting from cancer treatment. Int J Radiat Oncol Biol Phys 1995;31:1237-48. [Crossref] [PubMed]
- Jung J, Yoon SM, Kim SY, et al. Radiation-induced liver disease after stereotactic body radiotherapy for small hepatocellular carcinoma: clinical and dose-volumetric parameters. Radiat Oncol 2013;8:249. [Crossref] [PubMed]
- Cárdenes HR, Price TR, Perkins SM, et al. Phase I feasibility trial of stereotactic body radiation therapy for primary hepatocellular carcinoma. Clin Transl Oncol 2010;12:218-25. [Crossref] [PubMed]
- Andolino DL, Johnson CS, Maluccio M, et al. Stereotactic body radiotherapy for primary hepatocellular carcinoma. Int J Radiat Oncol Biol Phys 2011;81:e447-53. [Crossref] [PubMed]
- Tse RV, Hawkins M, Lockwood G, et al. Phase I study of individualized stereotactic body radiotherapy for hepatocellular carcinoma and intrahepatic cholangiocarcinoma. J Clin Oncol 2008;26:657-64. [Crossref] [PubMed]
- Klein J, Korol R, Lo SS, et al. Stereotactic body radiotherapy: an effective local treatment modality for hepatocellular carcinoma. Future Oncol 2014;10:2227-41. [Crossref] [PubMed]
- Bae SH, Kim MS, Cho CK, et al. Predictor of severe gastroduodenal toxicity after stereotactic body radiotherapy for abdominopelvic malignancies. Int J Radiat Oncol Biol Phys 2012;84:e469-74. [Crossref] [PubMed]
- Thomas TO, Hasan S, Small W Jr, et al. The tolerance of gastrointestinal organs to stereotactic body radiation therapy: what do we know so far? J Gastrointest Oncol 2014;5:236-46. [PubMed]
- Sorafenib-RT Treatment for Liver Metastasis (SLIM). Clin Regist database. Updated April 10, 2017. Available online: http://clinicaltrials.gov/ct2/show/NCT00892424
- Bentzen SM, Constine LS, Deasy JO, et al. Quantitative Analyses of Normal Tissue Effects in the Clinic (QUANTEC): An Introduction to the Scientific Issues. Int J Radiat Oncol Biol Phys 2010;76:S3-9. [Crossref] [PubMed]
- Lausch A, Sinclair K, Lock M, et al. Determination and comparison of radiotherapy dose responses for hepatocellular carcinoma and metastatic colorectal liver tumours. Br J Radiol 2013;86:20130147. [Crossref] [PubMed]
- Campbell A, Sivakumaran T, Davidson M, et al. Mathematical modeling of liver metastases tumour growth and control with radiotherapy. Phys Med Biol 2008;53:7225-39. [Crossref] [PubMed]
- Rule W, Timmerman R, Tong L, et al. Phase I dose-escalation study of stereotactic body radiotherapy in patients with hepatic metastases. Ann Surg Oncol 2011;18:1081-7. [Crossref] [PubMed]
- Lee MT, Kim JJ, Dinniwell R, et al. Phase i study of individualized stereotactic body radiotherapy of liver metastases. J Clin Oncol 2009;27:1585-91. [Crossref] [PubMed]
- Hoyer M, Roed H, Traberg Hansen A, et al. Phase II study on stereotactic body radiotherapy of colorectal metastases. Acta Oncol 2006;45:823-30. [Crossref] [PubMed]
- Chang DT, Swaminath A, Kozak M, et al. Stereotactic body radiotherapy for colorectal liver metastases: A pooled analysis. Cancer 2011;117:4060-9. [Crossref] [PubMed]
- Bujold A, Massey CA, Kim JJ, et al. Sequential phase I and II trials of stereotactic body radiotherapy for locally advanced hepatocellular carcinoma. J Clin Oncol 2013;31:1631-9. [Crossref] [PubMed]
- Sanuki N, Takeda A, Mizuno T, et al. Tumor response on CT following hypofractionated stereotactic ablative body radiotherapy for small hypervascular hepatocellular carcinoma with cirrhosis. AJR Am J Roentgenol 2013;201:W812-20. [Crossref] [PubMed]
- Vickress J, Lock M, Lo S, et al. A multivariable model to predict survival for patients with hepatic carcinoma or liver metastasis receiving radiotherapy. Future Oncol 2017;13:19-30. [Crossref] [PubMed]
- McCammon R, Schefter TE, Gaspar LE, et al. Observation of a Dose-Control Relationship for Lung and Liver Tumors After Stereotactic Body Radiation Therapy. Int J Radiat Oncol Biol Phys 2009;73:112-8. [Crossref] [PubMed]
- Chen QS, Weinhous MS, Deibel FC, et al. Fluoroscopic study of tumor motion due to breathing: Facilitating precise radiation therapy for lung cancer patients. Med Phys 2001;28:1850-6. [Crossref] [PubMed]
- Stevens CW, Munden RF, Forster KM, et al. Respiratory-driven lung tumor motion is independent of tumor size, tumor location, and pulmonary function. Int J Radiat Oncol Biol Phys 2001;51:62-8. [Crossref] [PubMed]
- George R, Vedam SS, Chung TD, et al. The application of the sinusoidal model to lung cancer patient respiratory motion. Med Phys 2005;32:2850-61. [Crossref] [PubMed]
- Vedam SS, Kini VR, Keall PJ, et al. Quantifying the predictability of diaphragm motion during respiration with a noninvasive external marker. Med Phys 2003;30:505-13. [Crossref] [PubMed]
- Suramo I, Päivänsalo M, Myllylä V. Cranio-caudal movements of the liver, pancreas and kidneys in respiration. Acta Radiol Diagn (Stockh) 1984;25:129-31. [Crossref] [PubMed]
- Shimizu S, Shirato H, Xo B, et al. Three-dimensional movement of a liver tumor detected by high-speed magnetic resonance imaging. Radiother Oncol 1999;50:367-70. [Crossref] [PubMed]
- Park JC, Park SH, Kim JH, et al. Liver motion during cone beam computed tomography guided stereotactic body radiation therapy. Med Phys 2012;39:6431. [Crossref] [PubMed]
- Case RB, Sonke JJ, Moseley DJ, et al. Inter- and Intrafraction Variability in Liver Position in Non-Breath-Hold Stereotactic Body Radiotherapy. Int J Radiat Oncol Biol Phys 2009;75:302-8. [Crossref] [PubMed]
- Keall PJ, Mageras GS, Balter JM, et al. The management of respiratory motion in radiation oncology report of AAPM Task Group 76. Med Phys 2006;33:3874-900. [Crossref] [PubMed]
- Brock KK. Imaging and Image-Guided Radiation Therapy in Liver Cancer. Semin Radiat Oncol 2011;21:247-55. [Crossref] [PubMed]
- Baron RL. Understanding and optimizing use of contrast material for CT of the liver. AJR Am J Roentgenol 1994;163:323-31. [Crossref] [PubMed]
- Bipat S, van Leeuwen MS, Comans EF, et al. Colorectal Liver Metastases: CT, MR Imaging, and PET for Diagnosis—Meta-analysis. Radiology 2005;237:123-31. [Crossref] [PubMed]
- Milot L, Guindi M, Gallinger S, et al. MR imaging correlates of intratumoral tissue types within colorectal liver metastases: a high-spatial-resolution fresh ex vivo radiologic-pathologic correlation study. Radiology 2010;254:747-54. [Crossref] [PubMed]
- Pan T, Lee TY, Rietzel E, et al. 4D-CT imaging of a volume influenced by respiratory motion on multi-slice CT. Med Phys 2004;31:333-40. [Crossref] [PubMed]
- Rietzel E, Pan T, Chen GT. Four-dimensional computed tomography: Image formation and clinical protocol. Med Phys 2005;32:874-89. [Crossref] [PubMed]
- Keall PJ, Starkschall G, Shukla H, et al. Acquiring 4D thoracic CT scans using a multislice helical method. Phys Med Biol 2004;49:2053-67. [Crossref] [PubMed]
- Vedam SS, Keall PJ, Kini VR, et al. Acquiring a four-dimensional computed tomography dataset using an external respiratory signal. Phys Med Biol 2003;48:45-62. [Crossref] [PubMed]
- Keall PJ, Vedam SS, George R, et al. Respiratory regularity gated 4D CT acquisition: concepts and proof of principle. Australas Phys Eng Sci Med 2007;30:211-20. [Crossref] [PubMed]
- Xhaferllari I, Chen JZ, MacFarlane M, et al. Dosimetric planning study of respiratory-gated volumetric modulated arc therapy for early-stage lung cancer with stereotactic body radiation therapy. Pract Radiat Oncol 2015;5:156-61. [Crossref] [PubMed]
- Fitzpatrick MJ, Starkschall G, Antolak JA, et al. Displacement-based binning of time-dependent computed tomography image data sets. Med Phys 2006;33:235. [Crossref] [PubMed]
- Low DA, Nystrom M, Kalinin E, et al. A method for the reconstruction of four-dimensional synchronized CT scans acquired during free breathing. Med Phys 2003;30:1254-63. [Crossref] [PubMed]
- McClelland JR, Blackall JM, Tarte S, et al. A continuous 4D motion model from multiple respiratory cycles for use in lung radiotherapy. Med Phys 2006;33:3348-58. [Crossref] [PubMed]
- Wink N, Panknin C, Solberg TD. Phase versus amplitude sorting of 4D-CT data. J Appl Clin Med Phys 2006;7:77-85. [Crossref] [PubMed]
- Kleshneva T, Muzik J, Alber M. An algorithm for automatic determination of the respiratory phases in four-dimensional computed tomography. Phys Med Biol 2006;51:N269-76.
- Lu W, Nystrom MM, Parikh PJ, et al. A semi-automatic method for peak and valley detection in free-breathing respiratory waveforms. Med Phys 2006;33:3634-6. [Crossref] [PubMed]
- Rietzel E, Chen GT. Improving retrospective sorting of 4D computed tomography data. Med Phys 2006;33:377-9. [Crossref] [PubMed]
- Ehrhardt J, Werner R, Säring D, et al. An optical flow based method for improved reconstruction of 4D CT data sets acquired during free breathing. Med Phys 2007;34:711-21. [Crossref] [PubMed]
- Zeng R, Fessler JA, Balter JM, et al. Iterative sorting for four-dimensional CT images based on internal anatomy motion. Med Phys 2008;35:917-26. [Crossref] [PubMed]
- Zhang Q, Pevsner A, Hertanto A, et al. A patient-specific respiratory model of anatomical motion for radiation treatment planning. Med Phys 2007;34:4772-81. [Crossref] [PubMed]
- Carnes G, Gaede S, Yu E, et al. A fully automated non-external marker 4D-CT sorting algorithm using a serial cine scanning protocol. Phys Med Biol 2009;54:2049-66. [Crossref] [PubMed]
- Chi PC, Balter P, Luo D, et al. Relation of external surface to internal tumor motion studied with cine CT. Med Phys 2006;33:3116-23. [Crossref] [PubMed]
- Gaede S, Carnes G, Yu E, et al. The use of CT density changes at internal tissue interfaces to correlate internal organ motion with an external surrogate. Phys Med Biol 2009;54:259-73. [Crossref] [PubMed]
- Balter JM, Ten Haken RK, Lawrence TS, et al. Uncertainties in CT-based radiation therapy treatment planning associated with patient breathing. Int J Radiat Oncol Biol Phys 1996;36:167-74. [Crossref] [PubMed]
- Gagné IM, Robinson DM. The impact of tumor motion upon CT image integrity and target delineation. Med Phys 2004;31:3378. [Crossref] [PubMed]
- Berbeco RI, Neicu T, Rietzel E, et al. A technique for respiratory-gated radiotherapy treatment verification with an EPID in cine mode. Phys Med Biol 2005;50:3669-79. [Crossref] [PubMed]
- Rietzel E, Chen GT, Choi NC, et al. Four-dimensional image-based treatment planning: Target volume segmentation and dose calculation in the presence of respiratory motion. Int J Radiat Oncol Biol Phys 2005;61:1535-50. [Crossref] [PubMed]
- Martin S, Rodrigues G, Patil N, et al. A multiphase validation of atlas-based automatic and semiautomatic segmentation strategies for prostate MRI. Int J Radiat Oncol Biol Phys 2013;85:95-100. [Crossref] [PubMed]
- Beddar AS, Briere TM, Balter P, et al. 4D-CT imaging with synchronized intravenous contrast injection to improve delineation of liver tumors for treatment planning. Radiother Oncol 2008;87:445-8. [Crossref] [PubMed]
- Loyer EM, Chin H, DuBrow RA, et al. Hepatocellular carcinoma and intrahepatic peripheral cholangiocarcinoma: enhancement patterns with quadruple phase helical CT- a comparative study. Radiology 1999;212:866-75. [Crossref] [PubMed]
- Silverman PM. Liver metastases: Imaging considerations for protocol development with Multislice CT (MSCT). Cancer Imaging 2006;6:175-81. [Crossref] [PubMed]
- Kanematsu M, Kondo H, Goshima S, et al. Imaging liver metastases: Review and update. Eur J Radiol 2006;58:217-28. [Crossref] [PubMed]
- Landberg T, Chavaudra J, Dobbs H. ICRU Report 50: Prescribing, Recording, and Reporting Photon Beam Therap. Bethesda, MD, USA. 1993.
- International Commission on Radiation Units and Measurements. ICRU Report 62: Prescribing, Recording, and Reporting Photon Beam Therapy (Supplement to ICRU Report 50). Bethesda, MD, USA. 1999.
- Burman C, Kutcher GJ, Emami B, et al. Fitting of normal tissue tolerance data to an analytic function. Int J Radiat Oncol Biol Phys 1991;21:123-35. [Crossref] [PubMed]
- Dawson LA, Normolle D, Balter JM, et al. Analysis of radiation-induced liver disease using the Lyman NTCP model. Int J Radiat Oncol Biol Phys 2002;53:810-21. [Crossref] [PubMed]
- Ben-Josef E, Normolle D, Ensminger WD, et al. Phase II trial of high-dose conformal radiation therapy with concurrent hepatic artery floxuridine for unresectable intrahepatic malignancies. J Clin Oncol 2005;23:8739-47. [Crossref] [PubMed]
- Dawson LA, Eccles C, Craig T. Individualized image guided iso-NTCP based liver cancer SBRT. Acta Oncol 2006;45:856-64. [Crossref] [PubMed]